Now we just need this evaluated to see how successful it is in recovering hand function. At least this is totally external, no glove to impossibly get on over spastic fingers.
Soft Rehabilitation Actuator With Integrated Post-stroke Finger Spasticity Evaluation
- 1Department of Biomedical Engineering, Faculty of Engineering, The Chinese University of Hong Kong, Shatin, Hong Kong
- 2Department of Surgery, The Chinese University of Hong Kong, Shatin, Hong Kong
Strokes cause severe impairment of hand function because of the spasticity in the affected upper extremities. Proper spasticity evaluation is critical to facilitate neural plasticity for rehabilitation after stroke. However, existing methods for measuring spasticity, e.g. Modified Ashworth Scale (MAS), highly depends on clinicians’ experiences, which are subjective and lacks quantitative details. Here, we introduce the first rehabilitation actuator that objectively reflects the condition of post-stroke finger spasticity. The actuator is 3D printed with soft materials. By considering the finger and the actuator together, the spasticity, i.e. stiffness, in finger is obtained from the pressure–angle relationship. The method is validated by simulations using finite element analysis (FEA) and experiments on mannequin fingers. Furthermore, it is examined on four stroke subjects and four healthy subjects. Results show the finger stiffness increases significantly from healthy subjects to stroke subjects, particularly those with high MAS score. For patients with the same MAS score, stiffness variation can be a few times. With this soft actuator, a hand rehabilitation robot that may tell the therapeutic progress during the rehabilitation training is readily available.
Introduction
Stroke has been the leading cause of disability. Every 40 s there will be a new stroke case (Emelia et al., 2017). Finger flexor spasticity, a motor disorder which results from impaired reflex function and causes involuntary muscle contraction, is one of the common disabling symptoms after stroke (Dietz and Sinkjaer, 2007). The onset of spasticity can occur within the first few days or weeks to around 30% of patients (Mayer and Esquenazi, 2003). The hands of patients who are affected by significant levels of post-stroke flexor spasticity remain tightly clenched due to the increased muscle tone in finger flexors, which is also believed to be the underlying reason of the difficulty in extending the fingers for stroke patients (Marciniak, 2011). In other words, this spasticity increases stiffness of the finger joints and furthermore leads to a decrease of their range-of-motion (ROM), creating severe reduction to hand function (Sadarangani et al., 2017). As spasticity and motor recovery are both related to neural plasticity after stroke, to lead targeted rehabilitation interventions, it is suggested that clinicians should decide the best treatment option for each patient based on their spasticity condition (Hong et al., 2018; Jeanette et al., 2019). In current clinical practice, primary measures (Bohannon and Smith, 1987; Copley and Kuipers, 1999; Gregson et al., 1999; Mackey et al., 2004) such as Modified Ashworth Scale (MAS), Modified Tardieu Scale (MTS), Tone Assessment Scale, and King’s Hypertonicity Scale are widely used to grade the resistance on the joints during passive soft tissue stretching (Thibaut et al., 2013). Such clinical scores quickly generate insights for therapists about the change in passive stiffness opposing the rotation of the examined joints. Nevertheless, result subjectivity and rater reliability have been continuously questioned by researchers since the measurement is completely dependent on clinicians’ experience (Damiano et al., 2002; Fleuren et al., 2010). Consistency of the results cannot be ensured among different assessors even if adequate training is provided (Pandyan et al., 1999). Therefore, there is a need of a procedure to objectively quantify finger joint stiffness for assessing hand function after stroke.
Various simple mechatronic devices are designed for standalone finger joint stiffness measurement after stroke (Milner and Franklin, 1998; Kamper and Rymer, 2000; Angelo et al., 2006; Yap et al., 2017). During the measurement, the forearm of patients is vertically clamped to a table for coupling the rotation of metacarpophalangeal (MCP) joints of all digits to a servomotor. To compute the joint stiffness, the angle and torque of MCP joints are measured by a torque transducer during rotation. However, since the stiffness of MCP joint cannot be individually assessed across each digit, it remains difficult to systematically evaluate the impairment of hand function based on the condition of each finger. Furthermore, due to the bulky size of all components (mounting platform of forearm, servomotor, and torque transducer), the devices are hard to be used to further examine the stiffness of other joints, e.g. proximal interphalangeal (PIP) joints that the stiffness is comparable to MCP joints after contracture (Prosser, 1996; Tang et al., 2019), in each finger. Additional robotic systems are required to independently examine the joint contracture for each digit in order to standardize the quantification of the hand function impairment level for better interventions guidance.
Recently, robotic hands have been proposed as wearable devices that facilitate movement of each individual finger in both flexion and extension direction. Susanto et al. have introduced the hand exoskeleton robot for active individual finger control via joint moment sensing (Tong et al., 2010; Evan et al., 2015). Therapeutic effect of the device has been demonstrated in the post-stroke rehabilitation training. To reflect the improvement of hand function, angle and torque of MCP and PIP joints are measured across each individual finger for examining the finger individuation (Wolbrecht et al., 2018), which is an important and comprehensive target for rehabilitative hand training. One major limitation of these robotic hands is shown in the bulkiness of mechanical linkages that translate finger movement to linear actuators, making them unsuitable for patients wearing them to perform activities of daily living (ADL). To address this problem in the rigid mechanical robotic systems, soft robots have been developed (Laschi and Cianchetti, 2014). Yap et al. (2017) and Cappello et al. (2018) have developed the robotic hands by the utilization of soft bending actuators to their bi-directional robotic gloves (Hong et al., 2017), which further reduced their weight and facilitated the performance of ADL, e.g. grasp of a bottle, using the soft robotic gloves. Nevertheless, since the evaluation of finger joint stiffness is not integrated with these actuators, the continuous stiffness measurement to indicate the performance of hand function using existing soft robotic hands is still lacking, and only pre-determined training exercises can be offered to patients regardless of their finger spasticity conditions. Since the level of finger spasticity varies during the ADL and therapeutic training, it is important to know the finger joint stiffness in real-time (Thibaut et al., 2013). By integrating the function of individual joint stiffness sensing to rehabilitation training, the robotic system could therefore offer optimum training exercises and assistance with ADL to stroke patients.
In this study, we propose the 3D printed soft-elastic composite actuator (SECA) that adopted to our soft robotic hand (Figure 1A) for facilitating both flexion and extension of spastic fingers during the performance of ADL and rehabilitation training (Heung et al., 2019b). SECA modeling is presented for the relationship between the input pressures and the bending angles according to the energy distribution inside the SECA and the spastic finger joints. Stiffness equations of spastic finger joints are derived from the pressure–angle relationship of SECA when worn on spastic fingers. In the experimental results, the bending angles of SECA with free bending and when placed on model compromised fingers are given, and the stiffness of the model fingers is further examined by the derived stiffness equations upon the actuation of 3D printed SECA, as well as finite element method (FEM) and analytical results for validation. Last, eight subjects (four in stroke and four in healthy condition) are recruited for the preliminary evaluation with the 3D printed SECA installed on index fingers, and the results of measured MCP and PIP joint stiffness are compared with the scores of MAS for reflecting the clinical potential of our method.
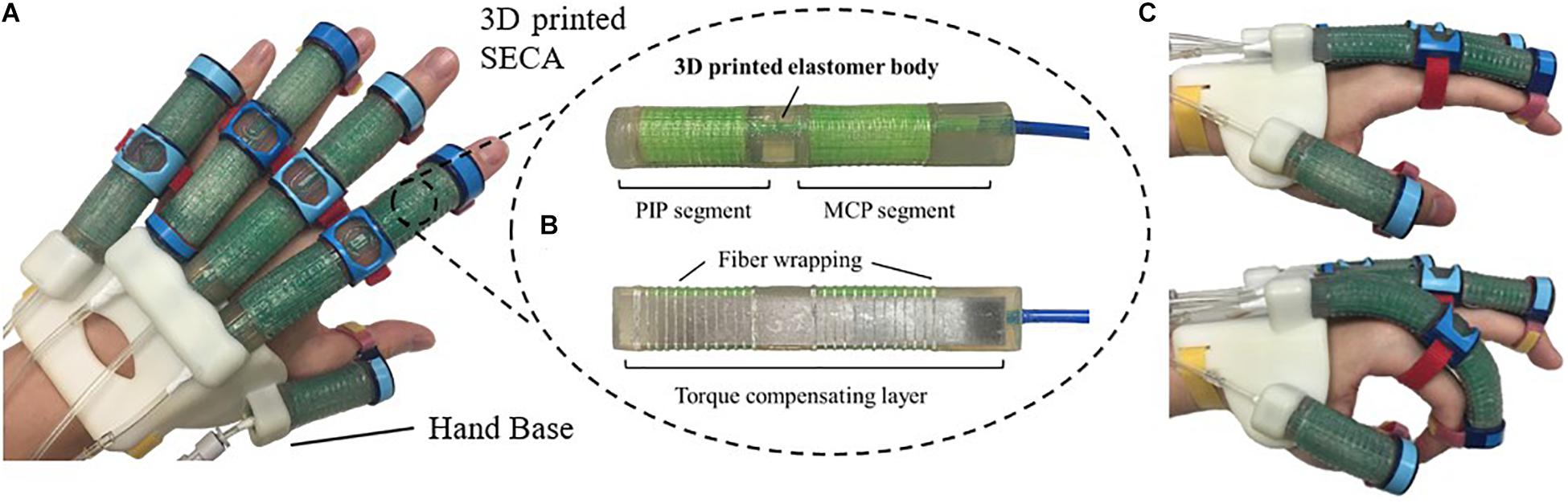
Figure 1. (A) Prototype of the 3D printed soft robotic hand for stroke rehabilitation and assistance of ADL. (B) Prototype of the 3D printed SECA. (C) Flexion and extension of the index finger (MCP and PIP joints) at 120 kPa on pneumatic actuation.
Significance
Stroke causes severe impairment of hand function. Spasticity and motor recovery are both related to neural plasticity after stroke. For rehabilitation planning, the level of finger spasticity that varies over different hand function tasks must be accurately evaluated. Unfortunately, existing spasticity indicators lack reliability, objectivity, consistency, and quantitative stiffness details. Moreover, there exists no hand rehabilitation devices that help objectively evaluate finger spasticity. Here, we introduce the first soft actuator for hand rehabilitation that quantifies spasticity of impaired fingers, i.e. stiffness, in real time. The method is validated with phantom fingers and eight subjects. With the stiffness information, optimal training tasks may be offered by our soft robotic system according to the varying spasticity conditions in fingers. Training outcome may also be improved by indicating the timely therapeutic progress, i.e. the decrease of joint stiffness, to patients for motivating them to achieve better hand function improvement.
3D Printed Soft Actuator
The 3D printed SECA (Figure 1B) presented in this work is composed of an elastomeric actuator body, fiber wrapping, and torque compensating layer, similar to our previous design by silicone molding (Heung et al., 2019b). The difference between SECA and traditional fiber-reinforced bending actuator (FRA) is that the SECA has a composite design with a torque compensating layer that can facilitate both flexion and extension on the same unit. Flexion and extension by the SECA are controlled under pressurization and depressurization, respectively. Meanwhile, FRA allows flexion under pressurization with a bottom strain limiting layer. Extension of FRA under depressurization can only be passively driven by the limited elasticity of elastomeric actuator body.
Metacarpophalangeal and PIP segments on the SECA correspond to actuation of the MCP and PIP joints of the human finger. When pressurized, the fiber wrapping around the actuator surface suppresses radial expansion, and the torque compensating layer further eliminates any axial elongation at the bottom, leaving only the upper section of the actuator to be elongated for bending the fingers. When depressurized, the torque compensating layer provides an assistive bending moment for the extension of fingers to the initial position (Figure 1C). The SECA weighs 21 g, which reduced the overall moment of inertia upon wearing and facilitated a more natural movement to fingers. Distal interphalangeal (DIP) joint is not addressed at all due to its small contribution to daily activities (around 15% of a functional grip) (Leibovic and Bowers, 1994).
In this study, we introduce the use of the latest industrial silicone 3D printer (ACEO Imagine, Burghausen, Germany) developed by ACEO® – WACKER Chemie AG, to directly 3D print the SECA. In the design of our 3D printed SECA, we select ACEO Silicone GP Shore A 30, an available 3D printed silicone rubber offered by ACEO® – WACKER Chemie AG, as the printing material for the elastomeric actuator body. The 3D printed silicone rubber offers sufficient elongation (450%) and tensile strength (6 MPa) to support large deformation and withstand high input pressure without creating any rupture, while the hardness (Shore A 30) is comparable to the silicone rubber of Dragon Skin Series (2019). Furthermore, a thin rigid elastic plate (A2 stainless steel plate) is used for the torque compensating layer, and directly reinforced to the bottom of SECA by the fiber wrapping.
No comments:
Post a Comment