Ask your competent? doctor how this will improve stroke recovery and EXACTLY WHAT THEY ARE DOING TO ENSURE RESEARCH OCCURS! Doing nothing I bet, so you really don't have a functioning stroke doctor , do you?
Recent Advances in Nanomaterials for Modulation of Stem Cell Differentiation and Its Therapeutic Applications
*
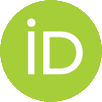
School of Integrative Engineering, Chung-Ang University, 84 Heukseuk-ro, Dongjak-gu, Seoul 06974, Republic of Korea
*
Author to whom correspondence should be addressed.
†
These authors contributed equally to this work.
Biosensors 2024, 14(8), 407; https://doi.org/10.3390/bios14080407
Submission received: 19 July 2024
/
Revised: 14 August 2024
/
Accepted: 20 August 2024
/
Published: 22 August 2024
(This article belongs to the Special Issue Functional Materials for Biosensing Applications)
Abstract
Challenges in directed differentiation
and survival limit the clinical use of stem cells despite their
promising therapeutic potential in regenerative medicine. Nanotechnology
has emerged as a powerful tool to address these challenges and enable
precise control over stem cell fate. In particular, nanomaterials can
mimic an extracellular matrix and provide specific cues to guide stem
cell differentiation and proliferation in the field of nanotechnology.
For instance, recent studies have demonstrated that nanostructured
surfaces and scaffolds can enhance stem cell lineage commitment
modulated by intracellular regulation and external stimulation, such as
reactive oxygen species (ROS) scavenging, autophagy, or electrical
stimulation. Furthermore, nanoframework-based and upconversion
nanoparticles can be used to deliver bioactive molecules, growth
factors, and genetic materials to facilitate stem cell differentiation
and tissue regeneration. The increasing use of nanostructures in stem
cell research has led to the development of new therapeutic approaches.
Therefore, this review provides an overview of recent advances in
nanomaterials for modulating stem cell differentiation, including
metal-, carbon-, and peptide-based strategies. In addition, we highlight
the potential of these nano-enabled technologies for clinical
applications of stem cell therapy by focusing on improving the
differentiation efficiency and therapeutics. We believe that this review
will inspire researchers to intensify their efforts and deepen their
understanding, thereby accelerating the development of stem cell
differentiation modulation, therapeutic applications in the
pharmaceutical industry, and stem cell therapeutics.
1. Introduction
Stem
cells are a versatile and promising class of cells that can self-renew
and differentiate into various specialized cell types, which makes them a
valuable tool for regenerative medicine and tissue engineering [1,2,3,4,5,6].
For example, stem cell therapy offers a new paradigm for individuals
with untreatable conditions, shifting the focus of treatment from solely
managing the disease to modulating immunopharmacological intervention
and regeneration [7,8,9,10,11].
In recent years, research on stem cells has produced increasing
evidence suggesting that stem cell transplantation is a highly effective
approach for treating neurological disorders, bone injuries, and
various diseases [12,13,14,15,16,17,18].
However, stem cell transplantation for clinical use has limited
effectiveness in producing mature specialized cells to replace damaged
cells [19,20,21].
In contrast, ex vivo differentiation of stem cells is known to have low
efficiency and poor survival when transplanted into the body [22,23].
Moreover, the ability to differentiate stem cells into specific cell
types of interest (e.g., bones, cartilage, and muscles) in a highly
selective and efficient manner remains a significant challenge [24,25,26,27,28].
To fully realize the therapeutic potential of stem cells in the field
of regenerative medicine, precise control of the fate of stem cells
should be addressed [29,30,31,32,33,34,35,36,37].
The surrounding matrix can significantly influence the development and
specialization of stem cells. Moreover, altering factors such as the
size, hydrophilicity, roughness, and organization of the cell attachment
surface can directly affect the cellular activity.
The
field of nanotechnology has made significant advancements in
influencing the stem cell behavior through the application of various
types of nanomaterials, including metal- and carbon-based ones and
nanoframeworks [38,39,40,41,42,43,44,45].
Metallic nanomaterials, such as gold nanoparticles (AuNPs), silver
nanoparticles (AgNPs), and other metal-based nanoparticles, have
recently gained significant attention owing to their wide range of
applications, including reactive oxygen species (ROS) scavenging,
autophagy, and thermoplasmonic regulation [46,47,48,49,50,51,52].
Carbon-based nanomaterials encompass fullerenes, carbon nanotubes
(CNTs), graphene and its derivatives, graphene oxide (GO), nanodiamonds
(NDs), and carbon-based quantum dots (CQDs) [53,54,55,56,57,58].
These materials have attracted significant interest because of their
distinct structural dimensions and remarkable properties in biomedical
fields, including cancer therapy and wearable device (reviewed elsewhere
[59,60]).
Finally, we focused the practicality of different nanomaterials in
regulating biomolecule delivery and facilitating stem cell
specialization, focusing on metal–organic frameworks (MOFs), zeolite
imidazolate frameworks (ZIFs), and upconversion nanoparticles (UCNPs) in
stem cell therapies [61,62].
In particular, nanomaterials with biodegradable and biocompatible
properties can be engineered to mimic the natural extracellular matrix
and provide specific chemical and physical cues to guide stem cell
differentiation and proliferation [63,64].
Recent
studies have investigated whether nanostructured surfaces and scaffolds
can enhance the proliferation, migration, and differentiation of stem
cells into specific lineages, such as osteogenic, adipogenic, or
neurogenic differentiation [65,66,67].
For instance, the surface topography, stiffness, and chemical
composition of nanomaterials have been shown to significantly impact
stem cell differentiation [68].
Moreover, nanomaterials can serve as delivery vehicles (e.g.,
metal–organic framework; self-assembled, peptide-based nanodrugs) for
various bioactive molecules, growth factors, and genetic materials,
which further enhances stem cell differentiation and tissue regeneration
[69,70,71].
This allows for the precise control and guidance of stem cell
differentiation, which is crucial for the development of stem-cell-based
therapies. The increased use of nanostructures in stem cell research
has led to the development of several new technologies, highlighting a
substantial demand for innovative therapeutic approaches.
This
review provides an overview of recent advances in nanomaterials across
metal-, carbon-, and peptide-based approaches, focusing on their
applications in enhancing stem cell differentiation and therapeutic
strategies (Figure 1).
It also focuses on strategies that commonly integrate nanostructures to
enhance differentiation and healing efficiency, along with descriptions
of common nanomaterial fabrication approaches used in stem cell
research. Finally, we conclude with a future perspective highlighting
clinical applications of stem cell therapy and advancement in
point-of-care treatments.
2. Metal-Based Stem Cell Differentiation Approaches and Therapeutics
Metallic
nanoparticles can guide stem cell fate and influence their
proliferation, migration, and differentiation. Nanomaterials such as
gold nanoparticles (AuNPs), silver nanoparticles (AgNPs), and other
metal-based nanoparticles have recently attracted considerable attention
for potent and broad applications such as medical carriers for in
regenerative medicine. These nanoparticles are used to direct stem cell
differentiation toward desired lineages, thereby enhancing the
therapeutic potential of these cells. The applications of metal-based
nanomaterials in stem cell research extend beyond directing
differentiation; they also show promise in stem cell tracking and
imaging [46,72,73].
Moreover, the integration of metal-based nanomaterials with advanced
biomaterials, such as hydrogels and diverse scaffolds, has further
expanded the therapeutic potential of these systems [74,75]. Here, we describe the use of various metal-based nanomaterials in controlling stem cell fate and biomedical applications. (Table 1).
2.1. Autophagy
Numerous
studies have demonstrated that the autophagy process is crucial for
preserving cellular homeostasis and enabling differentiation under
adverse conditions [85,86,87].
Additionally, impairment of cellular autophagy can lead to metabolic
disorders, such as accumulation of damaged proteins and/or organelles
and inability to clear protein aggregates, leading to compromised
stemness and regenerative capacity of stem cells [88,89].
Recent studies have shown that specific types of nanomaterials can be
internalized in cells and can accumulate in cellular compartments,
including endosomes, lysosomes, and autophagosomes, which activates
autophagy through their biological effects [90].
Accordingly, for therapeutic applications, nanoparticles can be used to
target the autophagy–lysosome system for stem cell rejuvenation. AuNPs
have emerged as the most preferred type of nanoparticle for use in
biological and pharmaceutical applications because of their unique
surface plasmon resonance and optical properties, as along with their
easily modifiable size, shape, functionalization, biocompatibility, and
regenerative ability [91,92,93].
Yin
et al. explored the potential of AuNPs to mitigate
inflammation-compromised osteogenic differentiation in the periodontal
ligament stem cells (PDLSCs) [94].
They evaluated the influence of AuNPs with different particle sizes on
the viability and osteogenic differentiation of the PDLSCs and their
inflammatory conditions (I-PDLSC). In terms of autophagy, the AuNP
treatment did not change the expression of LC3 II, an indicator of the
autophagic level, in I-PDLSCs during the early stage of osteogenic
differentiation. This was indicated by the lack of significant
difference in the LC3 II levels between the AuNPs and I-PDLSCs group.
Moreover, the AuNP treatment did not change the expression of LC3 II in
the I-PDLSCs during the early stage of osteogenic induction, as
indicated by the lack of a significant difference in the LC3 II levels
between the AuNPs and I-PDLSCs group. In contrast, the AuNP treatment
increased the autophagic flux in the I-PDLSCs, as indicated by the
significantly increased accumulation of LC3 II observed in the
AuNP-treated I-PDLSCs compared to the I-PDLSCs group at 12 or 24 h
during the early stage of osteogenic differentiation. Furthermore,
quantification of cellular autophagosomes revealed an elevated
proportion of RFP⁺-GFP⁺-LC3 puncta in the AuNP-treated I-PDLSCs
following treatment with Bafilomycin A1 (Baf), an autophagy inhibitor,
treated at 12 and 24 h during osteogenic differentiation. This is
consistent with the enhanced autophagic flux observed in these cells (Figure 2A).
However, the Baf treatment only enhanced the proportion of
autophagosomes (RFP⁺-GFP⁺-LC3 puncta) in the I-PDLSCs during osteogenic
differentiation. Moreover, the AuNP incubation increased the number of
accumulated FITC-labeled LC3 puncta in the I-PDLSCs compared to that in
the control I-PDLSCs group (Figure 2B).
The activation of transcription factor EB (TFEB), a master regulator of
the autophagy–lysosome system, and the expression of autophagy- or
lysosome-related genes in I-PDLSCs were also examined after the AuNP
treatment. The nuclear localization of the TFEB was enhanced in both the
I-PDLSCs and AuNP-treated I-PDLSCs after osteogenic induction. However,
the AuNP-treated I-PDLSCs exhibited greater nuclear TFEB levels during
the osteogenic differentiation than the control I-PDLSCs. Specifically,
the knockdown of the TFEB in the AuNP-treated I-PDLSCs inhibited the
AuNP-induced restoration of mineralized nodule formation (Figure 2C) and osteogenesis-related protein expression (Figure 2D).
In
organ transplantation, often the final therapeutic option in severe
diseases, survival is often limited by immunogenic rejection and/or
bacterial infection [95].
Utilizing gallium (Ga) coatings on biomedical devices can leverage
their antibacterial properties, affecting various bacteria [96].
Chen et al. developed a series of Mg-Ga-layered double hydroxide (LDH)
nanosheets on alkaline-treated titanium surfaces, which are composed of
positively charged brucite-like layers [97].
They fabricated Mg/Ga LDH sheets on the surface of alkali-heat-treated
titanium (AT) implants, which were subsequently calcinated to convert
them into Mg/Ga-layered double-oxide nanosheets with enhanced alkalinity
and stability. Their aim was to develop bone repair biomaterials and
investigate the relationship between autophagy and the pH of the local
microenvironment. Therefore, enhancing the alkalinity of implant
biomaterial surfaces may prove to be an effective strategy to enhance
autophagy and favor osteogenesis under osteoporotic conditions.
Through
biological investigations, researchers discovered that the coating
could promote autophagy by increasing the alkalinity of the surrounding
environment, thereby facilitating the osteogenic differentiation of
mesenchymal stem cells (MSCs) and inhibiting the bone resorption
activity of osteoclasts. Scanning electron microscopy (SEM) analysis
revealed the surface topographies of the samples (Figure 2E); the pure titanium and AT substrates exhibited relatively smooth surface morphologies. The Mg2+ and Ga3+
ions were incorporated into Mg-exchanged substrates through a
high-pressure hydrothermal process and subsequent calcination. The
resulting surface topography of the coatings displayed sheet-like
structures, with the sizes of these sheets progressively decreasing from
the AT-Mg to AT-Mg/Ga samples as the amount of Ga3+
increased. Further, to investigate the influence of pH, metal ions, and
substrate topography on autophagic activity, an autophagosome formation
test was performed on MSC under various conditions. The results (Figure 2F)
indicate that the presence of the Mg and Ga metal ions and the
topography of AT-Mg/Ga had no significant effect on the level of
autophagy. However, the expression level of LC3 II in the pH 8.5 group
was higher than in other groups. Interestingly, the addition of an
autophagy inhibitor eliminated the differences in ALP activity and
mineralization between the AT-Mg/Ga group and the control groups (Figure 2G).
Subsequently, osteoclastogenesis-related genes in RAW264.7 cells were
analyzed via qRT-PCR under the influence of the receptor activator of
the nuclear factor kappa-B ligand (RANKL) and macrophage
colony-stimulating factor (m-CSF). As shown in Figure 2H,
the AT-Mg/Ga group exhibited significantly lower expression of
osteoclastogenesis-related genes compared to the Ti and AT groups. To
evaluate osteoclast responses, researchers quantified the
tartrate-resistant acid phosphatase (TRAP) activity, a histochemical
marker for osteoclasts, in the RAW264.7 cells cultured on three sample
groups under the influence of RANKL and m-CSF. After 1 and 4 days of
culture, the TRAP activity was significantly lower in the AT-Mg/Ga group
than the control groups, particularly at day 4 (Figure 2I).
Additionally, while the AT-Mg/Ga sample exhibited only a few
multinuclear cells, numerous such cells were readily observed on the Ti
and AT groups (Figure 2J).
The results indicated that AT-Mg/Ga had significant potential to
inhibit the differentiation of the RAW264.7 cells in vitro and suppress
osteoclast generation and osteoclastic bone resorption in vivo under
osteoporotic conditions. This suggests that AT-Mg/Ga materials could be
applied in the development and research of functional orthopedic
implants for patients with osteoporosis.
In
conclusion, metal nanostructures, particularly AuNPs and GaNPs, have
proven to be crucial for preserving the potency and enhancing the
differentiation capacity of undifferentiated stem cells. Such
metal-based nanomaterials are extensively utilized to facilitate and
promote stem cell differentiation across diverse applications.
Figure 2.
(A) AuNP treatment enhanced autophagic activity in
inflammatory-conditioned periodontal ligament stem cells (I-PDLSCs)
during the early phase of osteogenic differentiation represented by
upregulated levels of LC3 II in the AuNP-treated I-PDLSCs and control
PDLSCs. (B) Confocal images of accumulated FITC-LC3 puncta per cell in the AuNP-incubated I-PDLSCs and I-PDLSCs. (C) Knockdown of TFEB abrogated the AuNP-mediated rescue of the osteogenic potential of I-PDLSC. (D) Osteogenic protein expression in the I-PDLSCs represented by the decrease in RUNX2 expression. (E) Topographic images of different Ti substrates using SEM. (F) Quantitative analysis of the LC3 expression in MSCs treated with various samples in normal DMEM media at 1 and 7 days. (G) Related qualitative ALP activity on various sample surfaces at 7 and 14 days. (H) Relative mRNA expression of osteoclastogenesis-related genes in the RAW264.7 cells grown on different substrates. (I) quantitative TRAP activities after incubation for 1 and 4 days. (J)
Confocal images of multinucleated cells on different substrates after
culturing for 4 days. The asterisks and number signs indicate p-values *p and # p < 0.05, ** p and ## p < 0.01, and *** p < 0.001. Reprinted with permission from [94]. Copyright 2022, Elsevier; reprinted with permission from [97]. Copyright 2022, Elsevier.
2.2. ROS Scavenger
Stem-cell-based
tissue regeneration has emerged as a promising approach for the
treatment of severe traumatic injuries and chronic wounds, including
cardiac repair, neurological trauma, bone defects, cartilage damage, and
diabetic foot complications. However, the low survival and impaired
function of implanted stem cells, largely due to excessive ROS in the
damaged microenvironment, have significantly limited their therapeutic
efficacy [98].
To address this challenge, engineered antioxidant nanomaterials have
been explored as potential strategies to enhance the resistance of stem
cells to oxidative stress and promote their regenerative capacity.
Accordingly, the development of ROS-scavenging nanostructures has
emerged as an intriguing approach to protect and regulate stem cells,
thereby facilitating tissue regeneration in high-ROS environments [99,100].
For instance, selenium, silver, and aluminum nanoparticles have been
utilized to alleviate oxidative stress and enhance the stemness and stem
cell differentiation. While recent progress has been made in developing
catalytic materials that can scavenge ROS, designing high-performance,
broad-spectrum ROS-scavenging materials with rapid enzyme-like catalytic
kinetics remains a significant challenge. Therefore, it is crucial to
develop suitable strategies to address the imbalanced valence states
during catalytic ROS scavenging and achieve reversible catalytic cycles
with high reaction activities.
Tian et al. described a novel strategy involving manganese-atom substitution to modulate the electronic structure of Co3O4 nanocrystals to enhance their multifaceted catalytic abilities to scavenge ROS [101]. This resulted in the Mn-Co3O4
material efficiently protecting human MSCs (hMSCs) from ROS-induced
damage, reversing apoptotic fates, and rescuing key cellular functions,
such as adhesion, spreading, proliferation, and osteogenic
differentiation. The performance of hMSCs treated with Mn-Co3O4 was comparable to that of the hMSCs cultured in standard medium (Figure 3A). The Mn-substituted Co3O4
materials were synthesized with varying Mn contents and denoted as
MC-0.4, MC-1.0, and MC-1.6. Because the MC-1.0 composition exhibited an
optimal catalytic ROS-scavenging activity, the subsequent analysis will
primarily focus on MC-1.0. All references to the Mn-Co3O4
materials pertain to the MC-1.0 composition and display spherical and
ultrasmall nanoscale morphologies with uniform dispersal (Figure 3B). Moreover, they present clear lattice fringes, corresponding to the lattice planes of Co3O4. To confirm the uniform crystal structures of Mn-Co3O4, X-ray diffraction (XRD) was performed, as shown in Figure 3C. The increased substitution of Mn atoms in Mn-Co3O4 results in a slight shift in the diffraction peaks toward lower diffraction angles compared to pristine Co3O4. This gradual Mn atom substitution indicates a slight disorder in the Co3O4 crystalline structure. Subsequently, the incorporation of Mn atoms into the Co3O4
crystal structure results in longer interatomic distances compared to
the native Co-Co bonds, as a consequence of the larger atomic radius of
Mn. The electron energy-loss spectroscopy further confirmed (Figure 3D) the homogeneous distribution of both Co and Mn atoms across the surface and edges of the Mn-substituted Co3O4
material. These findings suggest that the substituted Mn atoms are
evenly dispersed throughout the entire crystal lattice of the MC-1.0
composition. Furthermore, DPPH• is a commonly used reagent to assess the
free-radical scavenging capacity of biocatalysts. As shown in Figure 3E, the MC-1.0 catalyst also effectively removes DPPH radicals. To further investigate the potential of Mn-Co3O4
for stem-cell-based therapeutics, researchers systematically evaluated
its ability to regulate the fate of the hMSCs in high-ROS environments.
Subsequently, the proliferation of the hMSCs under high-ROS conditions
was studied to better understand the protective efficacy of MC-1.0 (Figure 3F). The H2O2-treated
hMSCs exhibited the lowest cell counts, indicating that the high
oxidative stress had impaired their proliferative capacity.
Interestingly, the H2O2 + hMSCs pretreated with MC-1.0 demonstrated more efficient cell proliferation than the H2O2-only
group. Meanwhile, the data suggest that MC-1.0 had a negligible effect
on cell proliferation compared to the control, further confirming the
good biocompatibility of the Mn-Co3O4 material. Previous studies have reported that excessive ROS can impair the osteogenic differentiation potential of the hMSCs [102].
Therefore, this study further investigated the differentiation
capabilities of the hMSCs under oxidative stress conditions. It was
found that the H2O2-induced suppression of
osteogenic gene expression could be rescued by the addition of MC-1.0.
Furthermore, immunofluorescence analyses revealed distinct differences
in the signal intensities of the osteogenic markers osteocalcin (OCN)
and osteopontin (OPN) between the H2O2-treated group and other groups (Figure 3G). These results suggest that H2O2 significantly inhibits the osteogenic differentiation of the hMSCs; however, the addition of MC-1.0 to the H2O2-containing
media can efficiently promote the expression of osteogenic genes in the
oxidative stress microenvironment. Collectively, these findings
indicate that the MC-1.0 nanomaterial can effectively protect the hMSCs
from ROS-induced damage and preserve their critical cellular functions,
including adhesion, spreading, proliferation, and differentiation.
Figure 3.
(A) Schematic illustrations of Mn-Co3O4 utilized as antioxidant nanostructures for regulating stem cell fates. (B) Crystal model of MC-1.0 which displays the optimized catalytic ROS-scavenging activity. (C) X-ray diffraction (XRD) analysis for the Mn-Co3O4 crystal structures. (D) Electron energy-loss spectroscopy (EELS) exhibiting uniformly distributed Co and Mn atoms on the surface. (E) Scavenging activities of Mn3O4, Co3O4, and MC-1.0 for DPPH radical. (F) Quantitative analysis of cell proliferation after the H2O2 treatment. (G) Relative fluorescence intensity of osteogenic markers. (H) Schematic diagram of the preparation of SOD-modified gold nanospheres (SOD@AuNS). (I) TEM and elemental mapping images of SOD@AuNS. (J)
Histological analysis of lipid accumulation (ORO) and calcium
deposition (ARS) by staining the MSCs, with and without labeling,
following adipogenic and osteogenic induction, respectively. (K) Quantitative analysis of each differentiation results. (L) Cell viabilities of the MSCs labeled with SOD@Au, SOD@AuNS, and MUA@AuNS. The asterisks indicate p-values * p < 0.05, ** p < 0.01, *** p < 0.001, **** p < 0.0001 and ns represents no significant difference. Reprinted with permission from [101]. Copyright 2022, Wiley Online Library; reprinted with permission from [103]. Copyright 2023, Elsevier.
A Mn-atom-substituted Co3O4
nanocrystalline structure and AuNPs can affect anti-inflammation and
remove ROS scavengers to protect the potential of the MSCs. Yu et al.
developed superoxide dismutase (SOD)-engineered AuNPs as a comprehensive
ROS scavenger. SOD is a crucial antioxidant enzyme that neutralizes
intracellular ROS and computed tomography (CT) contrast agent for
simultaneous protection and imaging tracking of MSCs. It was modified on
the surface of the AuNPs and then encapsulated within polyphosphazene
nanospheres (NS) (Figure 3H) [103].
This approach aimed to overcome the limited cell membrane penetration
and chemical instability of SOD to enhance the survival of MSCs in a
harsh inflammatory microenvironment through effective ROS elimination.
Further, transmission electron microscopy (TEM) analysis revealed that
the engineered SOD@AuNSs possessed a relatively uniform spherical
morphology with an average diameter of approximately 270 nm. Elemental
mapping further confirmed the presence of gold, phosphorus, nitrogen,
copper, and oxygen, which originated from the AuNPs, superoxide
dismutase enzyme, and polyphosphazene polymer backbone (Figure 3I). Moreover, the biocompatibility was also confirmed by CCK-8 analysis, maintained over 90% when co-incubated with SOD@AuNSs (Figure 3J).
Next, the intracellular localization of SOD@AuNPs in the MSCs was
investigated after nucleus staining. The results demonstrated that the
SOD@AuNPs were effectively internalized by the MSCs and predominantly
distributed within the cytoplasm. This suggests that the SOD@AuNSs can
provide feasible cellular contrast signals through stable labeling of
MSCs (Figure 3K).
Assessing the multipotency of the MSCs after labeling with
nanomaterials is crucial for their clinical application. For this
purpose, evaluating their ability to differentiate into osteogenic and
adipogenic lineages can provide insights into the maintenance of their
multipotent potential. As shown in Figure 3L,
the SOD@AuNS-labeled MSCs successfully differentiated into adipocytes
and osteocytes. Additionally, quantitative assays confirmed that there
was no significant difference in the differentiation between the labeled
and unlabeled MSCs. Furthermore, protecting stem cells from oxidative
stress using metal nanostructures and reversing their apoptotic fates to
rescue their critical functions is a promising approach for promoting
tissue regeneration. By regulating stem cell fate in microenvironments
with excessive ROS, this strategy can effectively advance stem-cell-
based therapeutics.
More at link.
No comments:
Post a Comment