Hope this moon shot works. But I'd rather they tackle known problems first. Like research that stops the 5 causes of the neuronal cascade of death in the first week. Much more likely to succeed.
Mesenchymal Stem Cell-Derived Extracellular Vesicle Therapy for Stroke: Challenges and Progress
- 1Department of Neurology, Samsung Medical Center, Sungkyunkwan University School of Medicine, Seoul, South Korea
- 2Translational and Stem Cell Research Laboratory on Stroke, Samsung Medical Center, Seoul, South Korea
- 3Medical Research Institute, Sungkyunkwan University School of Medicine, Seoul, South Korea
- 4Stem cell and Regenerative Medicine Institute, Samsung Biomedical Research Institute, Seoul, South Korea
Introduction
Stroke is the leading cause of physical disability among
adults. One-fourth to a half of stroke survivors are left with
significant disabilities. Stem cell therapy is considered a potential
regenerative strategy for patients with neurologic deficits. Adult stem
cells, such as mesenchymal stem cells (MSCs) may be a good option for
stroke therapy, as they secrete a variety of bioactive substances,
including trophic factors and extracellular vesicles (EVs, 30 nm−1 μm
sized circular membrane fragments shed from the cell surface) into the
injured brain, which is associated with enhanced neurogenesis,
angiogenesis, and synaptogenesis (1–5). In addition, MSCs are thought to play multiple roles, such as attenuating inflammation (6), reducing scar thickness (7), enhancing autophagy (8), and possibly replacing damaged cells (9),
in various brain diseases. Over the past 15 years, several randomized
stem cell therapy trials have been conducted in patients with ischemic
stroke (10–17),
which showed mixed results. Possible reasons for conflicting results
include, heterogeneous study populations (therefore requiring the
selection of optimal candidate patients), delayed treatment (thus
requiring an off-the shelf approach as soon as possible following a
stroke), the limited restorative potential of stem cell therapy
(especially in elderly patients with chronic illness), and a lack of
objective measurements for the assessment of efficacy in stem cell
therapy (5, 18).
It is widely accepted that MSCs exert their action via
paracrine effects via secretomes or EVs, rather than through
transdifferentiation to replace damaged neurons. Approximately 80% of
cells disappeared in the infarcted brain within several days after
transplantation with MSCs (19),
yet the effects of stem cells persisted for several weeks following
treatment. Our biodistribution study showed that MSCs exhibit a dynamic
release of EVs in the ischemic brain condition, and that systemic
administration of MSC-derived EVs led to a dose-dependent increase of
MSC EVs in the infarcted hemisphere (bypassing the lung and liver) and
functional improvement, suggesting that MSC EV therapy has a similar
functional outcome, yet an improved safety profile compared to MSC
administration (20).
This review presents the most recent advances in
MSC-derived EV therapy for stroke, focusing on the clinical application
of this strategy for stroke patients.
Biology and Function of Extracellular Vesicles
EV Biogenesis
EVs are a broad term that usually refers to
heterogeneous vesicles that are released from cells. EVs containing
cellular proteins, DNAs and RNAs of cells are classified into exosomes
(30–200 nm), microvesicles (200–1000 nm) and apoptosomes (1–10 μm)
depending on their size (21).
Among them, exosomes and microvesicles released from living cells, are
involved in many processes, such as proliferation, differentiation and
angiogenesis, and are known to act as a means of intercellular
communication (22–24).
Exosomes and microvesicles originate from the plasma membrane, and are formed through distinct mechanisms (Figure 1) (23).
The generation of microvesicles begins with the recruitment of
cytoplasmic proteins and nucleic acids by the endosomal sorting complex
required for transport (ESCRT)-dependent and independent pathways
{mediated by ADP ribosylation factor 6 [ARF6] and phospholipase D2
[PLD2]. Lipid flipping then occurs, and membrane budding takes place.
FIGURE 1
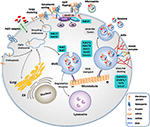
Microvesicles are a more
heterogeneous population and more sensitive to external stimulation than
exosomes. For example, an increase in the extracellular concentration
of ATP induces activation of the P2X7 receptor and consequential release
of microvesicles (21).
The production of exosomes begins with the membrane folding inward, the
creation of empty intraluminal vesicles (ILVs), and the maturation of
ILVs into multivesicular bodies (MVBs). They are released into the
extracellular space through fusion of MVBs and the plasma membrane by
small GTPases, such as RAB27A, RAB11, and RAB35, or by ESCRT (25).
Knowledge regarding EV biogenesis is essential for
understanding EV characteristics and for the development of EV
therapeutics. For example, activation of P2X7R by the
pathogen-associated molecular pattern (PAMP) or damage-associated
molecular pattern (DAMP) can induce membrane blebbing, or fusion of
MVBs. PLD2, which regulates lipids by degrading phosphatidylcholine into
choline and phosphatidic acid, and ARF6, which regulates membrane
trafficking and actin cytoskeleton remodeling, may play an important
role in endocytosis and exocytosis (23).
Studies have shown that overexpression of ARF6 increases the number of
exosomes released from the cells, whereas inhibition of ARF6 and PLD2
reduces the release of exosomes (26, 27).
Mechanisms of Action of Stem Cell-Derived EVs
Stem cell-derived EVs could play a critical role in the
exchange of information between stem cells and damaged cells and alter
the behavior of the target cells. Ischemia induces an increase in the
circulating or regional levels of EVs, and it has been identified that
EVs have their own function. Stroke triggers the mobilization of bone
marrow (BM) MSC-derived EVs in patients with severe stroke (28), and EVs released by ischemic stimulation have restorative capacity (20). In addition, EVs from ischemic tissue facilitated vasculogenesis in the ischemic limb model (29). EVs from ischemic muscles induce BM mononuclear cell differentiation into cells with an endothelial phenotype (29).
Formation of new neuronal cells and blood vessels are the
fundamental processes for the recovery after ischemic brain injury.
During acute phase of stoke, both ischemic/reperfusion injury and
inflammatory response are pivotal to the pathophysiology of ischemic
stroke. In addition, stroke patients are often elderly and have chronic
diseases, which may attenuate regenerative potential after stroke. As
shown in Table 1, regenerative potential could be enhanced by treatment of MSCs or MSC-derived EVs (67).
In addition, treatment of MSC-derived EVs in animal models of brain
diseases resulted in central and peripheral immunotolerance (63, 68). EVs harbor bioactive molecules and EVs secreted from stem cells carry more complex cargos than other cellular sources (69). Stem cell-derived EVs contain many molecules that may have therapeutic effects in stroke (70), such as microRNAs, proteins, and mitochondria (Table 1).
MicroRNAs, are a class of short, single-stranded, non-coding RNAs that
can be horizontally shuttled by EVs, and EVs-encapsulated proteins have
been implicated in the regulation of protective and restorative
processes (71). Beside microRNAs, MSC EVs may shuttle other genetic components, such as mRNAs (72).
In addition, damage to the mitochondria caused by tissue injury,
aggravates the severity of injury. Restoration of mitochondria
dysfunction, through stem cell-derived mitochondria transplantation via
EVs could potentially be an effective therapeutic strategy (66, 73).
TABLE 1
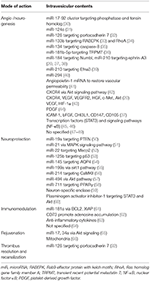
Advantages of Extracellualr Vesicles Over Stem Cells in Stroke
Allogeneic stem cells have many advantages over
autologous stem cells. Allogeneic MSCs are scalable from a manufacturing
perspective, with standardized procedures. The use of allogeneic MSCs
reduces the time required to obtain a sufficient number of cells (the
“off the shelf” approach). Through the application of allogeneic stem
cell therapy in the acute phase of stroke, both neurorestorative and
neuroprotective actions can be expected (74). In recent clinical trials of intravenous application of allogeneic stem cells (MultiStem® in patients with acute stroke, stem cells were applied within 24–48 h, following the onset of symptoms (16).
In addition, MSCs from younger healthy donors may differ in terms of
their proliferation and neurorestorative capacity, from those obtained
from elderly stroke patients with chronic illness (75).
However, conflicting results exist. Following serum
contact, allogeneic MSCs can be injured by complement, and the viability
of allogeneic MSCs after infusion is greatly reduced, compared with
autologous MSCs (76).
High mortality following intravenous transplantation of MSCs in animal
stroke models, and reports of pulmonary embolism following intravenous
injection of allogeneic adipose-derived MSCs have been accounted (77). MSC-related procoagulation status could be a possible explanation for such lethal pulmonary thromboembolism (78).
Lastly, cell diameters of MSCs are large, ranging from 15 to 30 μm,
which leads to passive arrest of MSCs in small diameter vessels, causing
vascular occlusion and reduction in cerebral blood flow, when
administered through intra-arterial routes, and also trapping in
systemic vessels such as the lungs, when administered systemically (the
first pass effect) (79–81).
The cell-free paradigm, using allogeneic MSC-derived EVs
could avoid such cell-related problems of allogeneic stem cell therapy.
EVs have low toxicity, high stability in the circulation, advantages in
scalable production and storage, and high transport efficiency to donor
cells (passing the blood-brain barrier [BBB] and avoiding the first pass
effect).
Applications of Stem Cell-Extracellular Vesicles for Treating Stroke
Preclinical Evidence of the Effects of EVs Derived From Various Stem Cells in Stroke
Xin et al. reported for the first time, that intravenous
application of MSC-derived EVs in a stroke rat model improved
neurological outcomes and increased angiogenesis and neurogenesis (47).
Other investigators have also demonstrated that stem cell-derived EVs
can be used for stroke therapy, as an alternative approach to stem cell
infusion methods (Table 2) (67, 87, 88).
In addition, several advances in EV-based strategy were introduced,
including: (a) the use of stem cells other than MSCs, such as EVs from
embryonic stem cells (ESCs), neural stem cells (NSCs), or induced
pluripotent stem cells (iPSC)-derived MSC/NSCs (64, 84, 86), (b) application of EVs via the intra-nasal approach (84),
(c) EV production other than conventional two-dimensional (2D) culture
methods to increase the production of EVs and regulate the contents of
EVs, e.g., 3D dynamic culture (37) and stimulation with ischemic brain extracts (20, 59), and (d) various EV isolation methods other than ultracentrifugation (82, 83, 85). Very recently, the effects of EVs on stroke has been tested in a large animal model of stroke (86).
TABLE 2
Recent Advances for EV Therapeutics
Various approaches are currently being employed to drive
the MSC secretome toward a more anti-inflammatory and regenerative
phenotype (88).
Because secretomes include a wide array of growth factors, cytokines,
and EVs, such approaches could also improve the efficacy of EV-based
therapy.
Firstly, conventional 2D cell culture systems often disregard the mechanical stimuli that significantly influence the intricate in vivo cellular microenvironment. Characteristics of EVs as well as phenotypes of stem cells could be affected by mechanical forces (89). For example, shear stress enhances the immune regulatory function of MSCs (90).
In addition, compared to conventional 2D cultured MSCs, MSCs cultured
in spheroid showed higher efficacy and safety profiles, and decreased
the expression of integrins, resulting in increased secretion of EVs (91, 92).
Cha et al. successfully amplified EV sections and therapeutic EV
contents (microRNAs and cytokines) from MSCs using a dynamic 3D culture
method, instead of using the conventional culture method (37).
In a traumatic brain injury model, EVs derived from MSCs cultured in 3D
scaffolds provided better outcomes than EVs from MSCs cultured in 2D
conditions, probably by promoting neurogenesis and angiogenesis (93).
Either native (decellularizing tissues) or synthetic 3D extracellular
matrix-based scaffolds can be utilized to provide a 3D environment for
cell attachment and growth (23).
Second, although MSC-derived EVs show promise in their
application for regenerative therapies, their use is often limited by
very low-yield conventional cell culture systems. Both microcarriers and
hollow-fiber bioreactors are currently used for large-scale cell
expansion of MSCs in the 3D environment (23) (89).
These methods may be particularly useful in MSC EV production, because
(a) large volumes of media would be required to get a sizable number of
EVs for clinical use, (b) viability of MSCs could be maintained by
continuous medium perfusion and avoiding metabolic by-product
accumulation in a bioreactor, without the use of serum, which contains a
large number of xenogeneic EVs, and (c) continuous processing, by
controlling culture medium flow in and out of a bioreactor, as is often
required because of the high advantages of reproducibility and safety of
the resulting EV products.
Third, preconditioning of sublethal stimuli can trigger
an adaptive response to further injury or damage. A wide variety of
molecules and culture methods can be used to prime MSCs and modify their
EVs. For example, Moon et al. showed that cultivation of MSCs with
either serum obtained from stroke patients, or treatment of ischemic
brain extracts on culture media, could activate restorative properties
of MSCs and the release of EVs, suggesting that signals from an ischemic
brain can affect the efficacy of MSCs and MSC-derived EVs and activate
the secretion of EVs from MSCs (20, 94). Similar findings were also reported by another research group (59). It is widely accepted that hypoxic conditions (i.e., 0.1–2% O2,
conditions similar to BM) were beneficial to MSCs and might stimulate
MSCs to exhibit adaptive responses. MSC culture in hypoxic conditions
with/without serum deprivation amplified EV sections, increased
therapeutic EV contents (e.g., microRNAs), and improved the EV efficacy
in tissue-injury models (48, 49, 56, 95). Inflammatory stimulation of MSCs renders release of EVs that have enhanced anti-inflammatory properties (96).
Fourth, as mentioned before, there have been advances in our current knowledge on the regulation of EV biogenesis (Figure 1). The modification of certain molecular pathways in EV biogenesis could lead to increased yield of EV production (23).
For example, activation of EV biogenesis during membrane blebbing (P2X7
receptor, phospholipase D2) or multivesicular body fusion with the
plasma membrane (Rab GTPase, SNARES) could increase EV secretion,
leading to an increased yield (23, 25, 97–100). In addition, genetic modification to overexpress certain therapeutic proteins or RNAs within EVs (Table 2)
could lead to an increased efficacy of EVs. For example, EVs harvested
from microRNA-133b-overexpressing MSCs improved neuronal plasticity and
functional recovery following stroke (33).
Furthermore, bioengineering techniques can be applied to produce
semi-synthetic artificial EVs to increase the expression of
functional/traceable molecules on EV surfaces/membranes or cargo, and
fully synthetic artificial EVs can be engineered to increase the yield
of EV production (101).
For example, “exosome-like nanovesicles,” which have morphological and
biochemical characteristics similar to EVs, can be made from cells
through cell membrane fragmentation (102).
Lastly, the source of EVs could be an important
determinant in the efficacy of stem cell-derived EVs in stroke. MSCs
have limited restorative potential in elderly patients. Similarly, MSC
EVs may have significant age-dependent differences in their cargo
contents (103). The transfer of EVs from young MSCs rejuvenated aged stem cells (65).
Fetal MSCs from amniotic fluid, cord blood, or Wharton's Jelly-derived
stem cells are reported to have intermediate cellular phenotypes between
ESCs/iPSC and MSC, in terms of expression patterns of both
marker/transcription factors of pluripotency and mesenchymal commitment,
as well as their broadly multipotent nature (104).
Although the use of ESC/iPSC-derived EV therapy may be safer than the
use of ESC/iPSC cell therapy, in terms of tumorigenicity, limited data
is available within the field of stroke and in human trials (64, 84). Therefore, fetal MSCs could be good sources of EVs in clinical application.
Clinial Applications of Extracellular Vesicle-Based Therapy
The effects of EV therapeutics have increasingly been reported in various animal disease/injury models (87).
However, only a few clinical studies on the effects of EV therapy have
been reported in humans. Kordelas et al. reported a case study, whereby
refractory graft-versus-host disease was treated with allogeneic MSC EVs
(105).
In this report, allogeneic MSCs were cultured in MSC conditioned media
and EVs were isolated by the polyethylene glycol (PEG) precipitation
method. EVs obtained from 4 × 107 MSCs were administered
repetitively four times. Clinical symptoms were improved, and no adverse
effects were observed. Katagiri et al. applied allogeneic MSC EVs via
local injection for alveolar bone regeneration in eight patients who
were diagnosed as needing bone augmentation prior to dental implant
placement, which revealed this method was safe and may have great
osteogenic potential (106). Lastly, Zhang et al. applied MSC EVs via intravitreal injection in five patients with refractory macular holes (107).
All three clinical studies are small case series, and although this
data suggests that MSC EVs are safe and may improve patient outcomes,
randomization trials are needed to investigate the efficacy and safety
of MSC EV therapy. No studies have examined the effects of stem
cell-derived EVs in stroke patients. Several phase I/II clinical trials
are ongoing to evaluate the application of EVs in cancer patients (108–110).
Considering MSC EVs are the therapeutically active
component of MSCs, are non-self-replicating and small sized, the
regulatory items required to produce EV fractions for clinical treatment
strategies could be less complicated than for MSC therapies. However,
compared to MSC therapy, clinical evaluation of EV therapeutics is still
at an early stage. Several issues must be considered and need to be
solved before the clinical application of EVs, including specific
guidelines targeting EV-based therapeutics, characterization, isolation,
and storage of EVs, quality control requirements, and in vivo analyses of EV. These issues were discussed precisely elsewhere (87, 111, 112), yet the following issues deserve mention in the application of EV for stroke patients.
First, the optimal time and mode of application of EVs
should be studied in stroke patients. Most recovery occurs in the first
few months following a stroke, with only minor additional measureable
improvements occurring thereafter. The levels of chemokines, trophic
factors, and related miRNAs increase markedly in the infarcted brain
during the acute phase of stroke but decrease over time. Such changes in
the brain microenvironment may greatly affect the biodistribution of
EVs, as well as the degree of recovery and neurogenesis/angiogenesis
after EV therapeutics in stroke patients.
Second, since EVs have many therapeutic components and
multiple modes of action, markers for potency and quality control should
be chosen carefully and should be measured during the freezing/thawing
procedures and storage period. EV therapeutics for stroke patients may
differ depending on the time (acute vs. chronic phase) of application.
For example, EV cargo components targeting neuroprotection and
immunomodulation are needed in patients with acute ischemic stroke,
while EV components targeting neurogenesis and angiogenesis are required
for neurorestoration in both acute and chronic stroke patients.
Differential markers for the potency of EVs (in vitro bioassays)
may be needed for patients with acute and chronic ischemic stroke. In
addition, customized stem cell-EV properties for stroke treatment are
needed. Given the heterogeneity of EVs in terms of cargo proteins and
RNAs, further studies are needed to increase the therapeutic components
of EVs for stroke patients in clinically feasible ways (33, 37, 56, 96, 113).
Lastly, the BBB is formed by the brain capillary
endothelium and excludes ~100% of large-molecule neurotherapeutics from
the brain and more than 98% of all small-molecule drugs (114).
As a result, compared with local application of EVs for topical
diseases or other systemic illnesses, stroke patients often require
large amounts of stem cells and stem cell-derived EVs. Therefore,
selection of culture media and isolation methods are particularly
important in EV therapeutics for stroke. Many different cell culture
media have been used in the production of EVs, including
serum-supplemented media, serum-free media, and EV-free/reduced
serum-supplemented media. Because a prior elimination of EVs from fetal
bovine serum is crucial, and commercial exosome/EV-depleted serum is
expensive and may be imperfect, various methods to deplete EVs are being
investigated, such as through the ultrafiltration method (115).
In addition, various techniques have currently been used for EV
isolation that include (but are not limited to) ultracentrifugation, PEG
precipitation, size exclusion chromatography, and tangential-flow
filtration. However, each method has advantages and disadvantages, and
there is no reliable method for isolation techniques for EVs (112). Recently, GMP-compatible methods for clinical scale production, purification, and isolation of EVs have been introduced (116).
Another important issue in improving the therapeutic effects of
EV-based therapy in stroke is BBB manipulation, which may enhance
endogenous repair mechanisms following stroke, by allowing entry of
paracrine factors (e.g., trophic factors and EVs) more easily to the
brain (117).
Conclusion and Future Perspectives
Cell therapy using EVs derived from stem cells could
represent a new, clinically feasible, and cell-free paradigm that would
avoid cell-related problems. Development of scientific research has just
begun in this stem cell-derived EV strategy when compared to that of
stem cell therapy. However, MSC-derived EV is rapidly expanding and
could be a promising approach for patients with severe stroke, as MSC
therapies have already been tested in preclinical and clinical trials
and EV-mediated therapy has unique advantages over MSC therapies in
stroke patients, in terms of biodistribution (cross the BBB and avoid
the first pass effect) and off-the-shelf approaches for acute ischemic
stroke.
There have been significant advances in the application
of stem cell-derived EVs for human diseases and our understanding of the
function and biogenesis of EVs. The efficacy of stem cell-derived EV
therapeutics will be improved with advances in our understanding of the
biology of stem cells and their EVs, together with advances in
techniques to modulate stem cell-derived EV characteristics, including
biotechnology and bioengineering. Future studies should focus on our
need for more well-designed preclinical studies of EV therapeutics in
animal models of stroke. Further studies should particularly focus on
biodistribution studies, optimal time/dose/mode of application, and
functional outcome measures with neuroimaging data. In addition, the
optimal cargo of EVs for EV therapies for stroke patients is unsettled.
Moreover, quality management of EVs and establishing standard operating
procedures for EV therapeutics are needed, as randomized trials of EV
for stroke patients are warranted.
No comments:
Post a Comment