Way beyond my pay grade so ask your doctor to decipher AND come up with stroke recovery protocols.
Regenerative Medicine Therapies for Targeting Neuroinflammation After Stroke
- Faculty of Biology, Medicine and Health, The University of Manchester, Manchester, United Kingdom
Introduction
Stroke is the second leading cause of death worldwide,
causing 6.2 million deaths each year accounting for 12 percent of all
deaths, with stroke-related illness, disability and early death set to
double by 2035 (1–3). A stroke occurs due to the disruption of blood flow to the brain by a bleed (hemorrhagic stroke) (4)
or a blockage (ischemic stroke), accounting for 15 and 85% of all
strokes respectively. While brain tissue ischemia occurs in ischemic
stroke, it remains unclear whether cerebral ischemia plays an important
role during hemorrhagic stroke. In both cases however, acute insult to
the brain leads to the formation of a cavity, or necrotic infarct and a
cavity (5).
Current therapy for ischemic stroke is limited to thrombolysis by
intravenous (i.v.) administration of recombinant tissue plasminogen
activator (rt-PA) given within 4.5 h of symptom onset, but is associated
with unwanted effects (6), or endovascular thrombectomy to physically remove the blood clot (7).
An endovascular thrombectomy can be performed as a complement to rt-PA,
but like thrombolysis, it has to be carried out within hours of stroke
onset and can be given to only a limited number of patients (7).
Ultimately, long-term rehabilitation therapy is available to most
stroke patients receiving daily sessions of motor functions, cognitive,
and speech language therapies, which has proven beneficial to regain
functional recovery to some extent (8).
The past decades has seen a large number of promising
therapeutic approaches in pre-clinical settings, however most have
failed to translate into clinical application. The reasons for these
failures remain largely unknown, and the Stroke Therapy Academic
Industry Roundtable (STAIR) (9) followed by STAIR meetings (10)
formulated several recommendations with the hope that ongoing
preclinical strategies could translate into successful therapies. One
main hypothesis behind the failure of clinical trials in stroke is that
current animal models are inadequate and simply do not replicate the
human pathology. As a result, current therapies remain exclusively
limited to thrombolysis and thrombectomy, and with an aging population
and access of developing countries to western lifestyle, the clinical
and socioeconomic impact of stroke and stroke-related complications is
on the rise, which is further potentiated by decreased post-stroke
mortality rate and patient care costs due to better rehabilitation and
clinical management procedures.
Despite the aforementioned interventions, no effective
treatment to promote brain tissue repair and restore brain functions
after stroke exist. Regenerative medicine is an emerging paradigm in the
field of stroke therapy that offers the potential to promote recovery
and regeneration of damaged neurovascular tissue at previously
unattainable levels. This builds on previous research into
neuroinflammation intertwined with the multidisciplinary research field
of regenerative medicine, utilizing biomaterials science and mechanical
engineering, as well as cell and gene therapies. This review focusses on
the use and limitations of anti-inflammatory regenerative medicine
therapies for stroke, with specific focus on the use of nanoparticles
(NPs), hydrogels, stem cells and gene-editing technologies to repair the
damaged brain tissue after stroke. The use of NPs and hydrogels in
particular has the potential to improve the administration of drug and
cell-based therapies through a controlled release of therapeutics at
appropriate doses, and therefore may enable the repurposing or revised
investigation of previously ineffective therapeutics.
Inflammation in Stroke
Shortly after vessel occlusion, post-ischemic
inflammation begins in the vascular compartment, peaking during the
first days after stroke onset (11).
Post-stroke inflammation response is characterized by blood-brain
barrier (BBB) disruption, infiltration of peripheral leukocytes,
activation of glial cells and the release of molecules known as
damage-associated molecular patterns (DAMPs) by injured and dying cells
(Figure 1).
Activated immune cells, triggered by DAMPs, produce inflammatory
cytokines, chemokines, and other cytotoxic mediators, leading to
exacerbation of cerebral ischemic injury (12).
During the sub-acute phase of stroke (weeks to months after stroke
onset) chronic inflammation and tissue remodeling (neurogenesis and
angiogenesis) take place, although ultimately repair is limited and a
fluid filled cavity develops, preventing full functional recovery (13, 14).
FIGURE 1
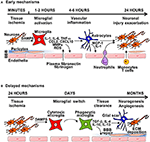
Early Mechanisms of Neuroinflammation
Under normal conditions, microglia, the main resident
immune cells in the brain, are primarily involved in monitoring
(surveying) the brain parenchyma, and are known to play an important
homeostatic role (15). In response to cerebral ischemia, microglia are rapidly activated, switching from a resting state to an activated state (16).
The inflammatory phenotype of early activated microglia is
characterized by the production of a variety of pro-inflammatory
cytokines including interleukin (IL)-1, IL-6, tumor necrosis factor
(TNF)-α, chemokines CCL2, and CXCL10, reactive oxygen species (ROS),
nitric oxide (NO), and proteolytic enzymes such as matrix
metalloproteinase (MMP)-9 and MMP-3 (17).
The release of cytokines and chemokines by activated
microglia/macrophage promotes recruitment of circulating immune cells to
damaged brain tissue that plays a critical role in pathophysiological
events following very acute stroke onset (18).
Such events are associated with BBB disruption and degradation of the
associated extracellular matrix (ECM), alongside activation of
perivascular astrocytes. After the onset of stroke, the BBB is rapidly
disrupted allowing uncontrolled entry of circulating molecules into the
brain parenchyma, and this disruption persists for days through the
acute and early subacute phases of stroke (19).
Clinically, BBB disruption leads to the development of hemorrhagic
transformation that is associated with worse stroke outcome (20),
and MMPs have been identified to play a key role in this process,
degrading all components of the ECM including laminin, collagen and
fibronectin, and the endothelial junction proteins claudin-5, occluding,
and zona occludens (ZO)-1 (21).
Opening of the BBB allows penetration of plasma-derived factors (plasma
fibronectin, fibrinogen) and inflammatory cells into the brain tissue,
causing edema and cell death (22).
Astrocytic death is a critical contributing step of BBB dysfunction in
stroke by decreasing expression of tight junction proteins (23).
Perivascular astrocytes express the passive water channel aquaporin 4
(AQP4) at astrocytic end-feet localized adjacent to the brain
endothelium that contributes to post-stroke edema (24).
In addition, astrocytes secrete chemokines such as monocyte
chemoattractant protein-1 (MCP-1), a critical mediator involved in
opening of the BBB after stroke (25). Astrocytes also synthesize a large array of cytokines (i.e., IL-1α, IL-1β, TNF-α) (26) that can directly trigger endothelial cell activation that contributes directly to BBB disruption (27).
Delayed Mechanisms of Neuroinflammation and Brain Repair
Cerebral ischemia also activates important delayed
endogenous repair processes such as BBB repair, neurogenesis, and
angiogenesis that are important for functional recovery and patient
rehabilitation in clinical settings, and evidence suggests that the
sub-acute phase of inflammation plays a key role in this process. The
anti-inflammatory phenotype of microglia exhibits neuroprotective and
anti-inflammatory effects during the delayed phase of post-stroke
inflammation, producing anti- inflammatory cytokines such as IL-10,
transforming growth factor (TGF)-β, IL-4, and IL-13, as well as scavenge
receptors, contributing to inhibiting inflammation and promoting tissue
repair mechanisms (28).
Of those, neurogenesis—known to take place in the sub granular zone of
the dentate gyrus of the hippocampus and in the sub ventricular zone
adjacent to the third ventricle (29)—is increased following experimental stroke (30), and is regulated by inflammatory mediators expressed during the acute phase of stroke (31).
However, neurogenesis in the adult mammalian brain has been debated,
with Sorrells and colleagues, reporting that human hippocampal
neurogenesis declines rapidly during early childhood and is rarely
detected in adult humans (32),
and the role of neurogenesis on functional recovery in human remains
unclear. In parallel, reactive astrocytes form a glial scar around the
ischemic infarct by 14 days (33).
Astrocytes initially proliferate and then migrate toward the site of
ischemic injury that becomes surrounded by multiple layers of reactive
astrocytes interspersed with activated microglia and a dense network of
ECM proteins such as laminin, fibronectin, and chondroitin sulfate
proteoglycans, resulting in the formation of a very tight glial scar (34). Angiogenesis is also a mechanism of recovery induced by inflammation after an ischemic stroke (35)
that is essential for the reoxygenation of post-ischemic brain tissue,
and is also an essential step for BBB repair, neurogenesis, and neuronal
synaptic plasticity (11).
Although there is clinical evidence to support that inflammation plays a key role in stroke (36–39),
the failure of anti-inflammatory strategies have raised hypotheses that
inflammation might not play a significant role in stroke
pathophysiology. This alterative hypothesis should be investigated more
extensively, and future anti-inflammatory therapies may prove to be
successful in the treatment of human stroke, providing more convincing
evidence for the role of inflammation in stroke pathophysiology.
Anti-inflammatory Strategies in Regenerative Medicine
Several strategies to prevent neuroinflammation and
modulate the immune response post-stroke have been studied in
experimental models and explored in clinical trials (Table 1). For instance, minocycline is a semi-synthetic tetracycline derivative (51).
In animal models of cerebral ischemia, minocycline administration
correlates with the reduction of several pro-inflammatory cytokines, as
well as ROS and NO (52).
A recent comprehensive systematic review and meta-analysis by Malhotra
and colleagues showed that minocycline is safe in ischemic stroke
patients and demonstrated efficacy and a neuroprotective role,
particularly in the acute ischemic stroke (53).
Furthermore, several approaches aimed at preventing neutrophil
infiltration, trafficking and/or activation have been explored;
experimental models using pharmacological agents to block leukocyte
adhesion and migration into the ischemic brain have shown promising
results (54, 55).
In particular, Fingolimod (FTY720) a sphingosine 1-phosphate receptor
(S1PR) modulator that prevents the egress of lymphocytes from lymph
nodes, has shown promise in preclinical models of stroke (56).
This is evident in a systematic review and meta-analysis which reports
that fingolimod reduced brain injury in eight out of nine studies (57).
There is an ongoing Phase 2 randomized, open-label trial of patients
receiving fingolimod within 72 h of ischemic stroke or spontaneous
intracerebral hemorrhage. Main outcome measures include NIHSS, BI, mRS,
GCS at d7, d14, d30, d90, brain MRI, and immune markers (58).
In patients with small- to moderate-sized deep primary supratentorial
ICH, administration of fingolimod reduced perihematomal edema,
attenuated neurologic deficits, and promoted recovery. The results for
ischemic stroke patients have not yet been reported. However, clinical
trials testing antibodies against adhesion molecules such as
intercellular cell adhesion molecule (ICAM)-1 or by administering
neutrophil inhibiting factor have been inconclusive (59, 60).
A recent phase 2 clinical trial, involving subcutaneous administration
of interleukin-1 receptor antagonist (IL-1Ra) in ischemic stroke has
shown promising results (61).
IL-1Ra is known to block actions of the pro-inflammatory cytokine IL-1,
which has a deleterious role in cerebral ischemia. IL-1Ra reduced
plasma inflammatory markers, which are known to be associated with worse
clinical outcome in ischemic stroke. Previous to this phase 2 clinical
trial, recombinant human IL-1Ra (anakinra) was administered as an i.v.
formulation, although it is no longer manufactured in this way (58).
Anakinra was evaluated in a UK Phase 2 randomized controlled trial
(RCT) in patients presenting within 6 h of acute stroke onset (39).
The drug was administered intravenously and there were no significant
safety concerns. Patients that received anakinra showed better clinical
outcome overall and had reduced neutrophil leukocytosis, plasma
C-reactive protein and plasma IL-6 levels during the 72 h infusion.
Statins inhibit the enzyme 3-hydroxy-3-methylglutaryl coenzyme A
reductase, lowering the level of low-density lipoprotein (LDL)
cholesterol in the blood (38). In a Phase 2 RCT, patients were treated with simvastatin (40 mg/day) within 24 h after the onset of acute ischemic stroke (62).
Serum TNF-α levels were marginally lower at day 3 in the
simvastatin-treated group, however no clinical outcomes were reported.
The safety and efficacy of simvastatin, in combination with rt-PA, is
currently being evaluated in the STARS07 trial (58).
Edaravone (MCI-186) is an antioxidant and free radical scavenger
evaluated in a Phase 2 clinical trial for the treatment of patients with
acute ischemic stroke within 24 h from the onset of symptoms (63).
MCI-186 was shown to be well-tolerated and safe. However, there were no
differences in clinical outcome measures after 1 year. The calcineurin
inhibitor cyclosporin A has shown efficacy in preclinical stroke models.
Studies reported a reduction of infarct size and inflammation as a
result of the drugs suppression of cytokines, T cell activation, and ROS
production (51, 64).
A Phase 2 clinical study investigating the effect of cyclosporin A
(single i.v. dose of cyclosporin A after i.v. thrombolysis within 4.5 h
of stroke onset) on MR infarct volume at day 30 has been completed, and
the results of this study has yet to be published (65).
TABLE 1
Past and current
anti-inflammatory therapies have not translated into a successful
clinical treatment for ischemic stroke. Regenerative medicine therapies
for stroke may alleviate some of these challenges by providing a
structural support, localizing therapy to the site of action, and/or
modulating endogenous regenerative cues to brain cells. The
multidisciplinary nature of the regenerative medicine approach improves
the likelihood of the development of an effective therapy for ischemic
stroke. When considering the aforementioned therapies, cyclosporin A,
edaravone, and IL-1Ra are the best candidate drugs for combination with
NP delivery as they are administered intravenously, thus encapsulation
into NPs would potentially improve blood circulation half-life and allow
for a more targeted and controlled drug delivery. NPs could be used for
the targeted therapeutic delivery of rt-PA, and Tadayon; colleagues
have studied the potential of silica-coated magnetic NPs as nanocarriers
for rtPA, showing promising results (66).
The CTX stem cell therapy developed by ReNeuron could be encapsulated
into a hydrogel, for injection into the ischemic brain, enabling
controlled delivery over time and better cell survival.
Demonstrating the efficacy of emerging regenerative
medicine therapies for ischemic stroke is important and challenging.
Magnetic resonance imaging (MRI) can be used to visualize and quantify
the infarct volume at multiple time points after stroke (67).
This method can be applied to both animal models and stroke patients,
and is arguably far more accurate than the determination of infarct
volume by immunohistostaining such as NeuN immunostaining or cresyl
violet staining (68, 69),
which cannot be achieved in humans. Stroke cavity size can also be
determined in order to evaluate whether a therapy is promoting repair
after stroke injury. For example, Wang and colleagues used cresyl violet
staining to quantify the cavity size in a mouse model of stroke (70).
As demonstrated by Zhang and colleagues, the effect of scaffold
implantation on the integrity of brain shape can be simply shown by
haematoxylin and eosin staining of rat brain sections or by extracting
and visually observing the whole brain (71). Regenerative medicine therapies may increase post-stroke neurogenesis (72), which can be assessed by doublecortin and NeuN/BrdU immunohistochemistry (73). Induction of post-stroke angiogenesis is considered to be beneficial and can be imaged by laminin immunohistochemistry (44).
Immunohistochemical staining for reactive astrocytes and activated
microglia is commonly used to determine whether a regenerative medicine
therapy is attenuating the inflammatory response after experimental
stroke (72). The translocator 18 kDa protein (TSPO) has been used in PET imaging studies to image glial activation and neuroinflammation (74). Recently, improved radioligands for this protein have been developed and approved for human imaging including (11C)PBR28, (18F)DPA-714, and (18F)FEPPA (75).
Ultra-small superparamagnetic particles of iron oxide (USPIO) can be
used for human imaging of monocyte/macrophage tracking, and have been
used successfully to study neuroinflammation in stroke patients (76). The PET ligand 11C-flumazenil
(FMZ), which targets GABA-A receptors, has been used for imaging
neuronal integrity in human stroke, with patients showing reduced FMZ
binding potential in ischemic brain regions (77).
Tracking of transplanted stem cells is essential to monitor safety and
efficiency of cell-based therapies. Citrate-coated superparamagnetic
iron oxide NPs have been used for in vivo stem cell tracking by MRI (78).
Zhu and colleagues reported a case of labeling human neural stem cells
(NSC) with superparamagnetic iron oxide NPs and tracking their survival,
migration, and distribution in a patient with brain trauma by MRI (79).
Additional promising imaging modalities for tracking stem cells include
nuclear imaging [Positron emission tomography (PET) and Single-photon
emission computed tomography (SPECT)] and optical imaging (80).
To evaluate changes in neurological function, animals can be subjected
to a variety of somatosensory, motor, learning, and memory tests before
and after surgery. For example, the rotarod test is widely used for
evaluating motor function and balance in rats and mice (81). In addition, the pole test and wire hanging test can be used to assess motor dysfunction after stroke (82).
Cognitive deficits including memory problems occur in human stroke
survivors thus memory tests have been developed for use in animals such
as water maze and passive avoidance task (83).
Tests that assess anxiety-like behavior in rodent models have been
developed, in order to address post-stroke anxiety that affects up to
40% of stroke survivors (84).
Popular tests for this include dark-light box, Vogel conflict test,
Geller- Seifter conflict test, elevated plus maze, and open field (81).
Anti-inflammatory Properties of Nanoparticles (NPs)
Nanoparticles (NPs) are colloidal carriers that can be of natural or synthetic origin and can vary in size from 1 to 1,000 nm (85). Natural NPs are primarily composed of molecules such as proteins (albumin), polysaccharides, or chitosan for instance (86).
Synthetic NPs are made from common polymers such as
poly(lactic-co-glycolic acid) (PLGA), poly(ethylenimine) (PEI),
polyesters poly(lactic acid) (PLA), or from inorganic agents such as
gold, silica or alumina (87).
NPs can be spherical, cubic and rod-like in shape, and they can have
negative, zwitterionic, or positive charge, affecting interactions with
biological substrates and the BBB (85).
NPs can be coated and functionalized with different types of ligands;
some are capable of mediating protein adsorption, others are able to
interact directly with the BBB, increase hydrophobicity, or are able to
improve blood circulation (88).
NPs are versatile drug delivery systems that can be used for the
targeted delivery of therapeutic agents into normally inaccessible
organs like the brain, and can also be used for the delivery of
lyophobic drugs (89).
Recently, it has been suggested that NPs could exert
potent anti-inflammatory effects by acting on ROS production, a key
process in stroke pathogenesis, since oxidative stress contributes to
the initiation of the post-ischemic inflammatory response (90). Recent work from Liu et al. (40)
has shown that polyethylene glycol-melanin NPs (PEG-MeNPs) exhibit
broad anti-oxidative properties against multiple toxic reactive oxygen
and nitrogen species (RONS) including superoxide ions (O2•−), hydrogen peroxide (H2O2), hydroxyl radical (•OH), peroxinitrite (ONOO–), and NO, highlighting their potential as a robust RONS scavenger (40).
Using a rat model of ischemic stroke, they showed that pre-injection of
PEG-MeNPs can significantly decrease ischemic brain injury. In vitro,
the NPs were shown to be anti-inflammatory, decreasing the expression
of cyclo-oxygenase 2 (COX-2), inducible nitric oxide synthase (iNOS),
TNF-α, and IL-1β in lipopolysaccharides(LPS)-stimulated macrophages.
Biocompatibility was assessed in vitro and in vivo, with
NPs demonstrating no obvious toxicity. Another type of NPs, retinoic
acid-loaded polymeric NPs (RA-NP) have been developed to modulate
microglial response toward an anti-inflammatory and somehow
neuroprotective phenotype (28).
RA-NP were internalized by murine N9 microglial cell line and inhibited
LPS-induced iNOS expression and NO release, whilst promoting arginase-1
and IL-4 production. Additionally, RA-NP effects on microglial
phenotype, promoted tissue viability and neuronal survival in
organotypic hippocampal slice cultures exposed to an inflammatory
stimulus. A new class of antioxidant NPs composed with hydrophilic
carbon clusters conjugated to poly(ethylene glycol), named PEG-HCCs,
have been recently developed (41).
They are effective at scavenging hydroxyl radical and have been found
to reduce infarct size when administered during the reperfusion period
after experimentally-induced stroke in rat (41).
Over the last decade, a variety of NPs (metal-based,
carbon-based, polymer-based, biological-based, and lipid-based) have
been investigated for their use in biomedical imaging (91).
In particular, the potential uses of iron oxide NPs as MRI contrast
agents has been an area of intense interest, and several types of these
particles, such as ferumoxytol, have been approved by the Food and Drug
Administration (USA) for their use in clinical diagnosis (92).
Europium-doped very small iron oxide NPs have been used to visualize
neuroinflammation with MRI combined with fluorescence microscopy (93).
In addition, there have been recent developments in molecular imaging
techniques using organic NPs and quantum dot applications for
visualizing in vivo molecular pathways (94, 95).
Clinically approved NPs are currently limited to SPECT imaging of
peripheral organs such as gastrointestinal tract, liver, and spleen (96).
Although nanotechnology has relieved many problems in biomedical
imaging, the clinical translation of many types of NPs is impeded by
fundamental limitations of human physiology (i.e., vessel pore size,
renal, and hepatic clearance), potential toxicity, and/or interference
with other medical tests. Hence, a refined NP design and extensive
toxicity studies will help facilitate the clinical translation of new
NPs that have unique advantages over conventional imaging agents.
Anti-inflammatory Properties of Hydrogels
Hydrogels are acellular polymeric networks that
replicate the intrinsic properties of the native ECM of the
neurovascular unit (NVU) (97, 98), and are therefore used commonly for in vitro cell culture and as an in vivo therapeutic tool. The polymeric constituents of hydrogels are termed as biopolymers, which are of natural or synthetic origin (97–99).
Natural hydrogels are formed of protein and polysaccharide biopolymers
that are either native constituents of the ECM, i.e., collagen, laminin
or hyaluronic acid, or can be structurally similar to the native ECM,
like alginate and gellan gum. Synthetic hydrogels are chemically
synthesized biopolymers—commonly peptide based—that can be designed to
assemble into an ECM-like conformation (also known as self-assembling
peptides). Hydrogels provide a supportive 3D microenvironment that is
similar to the native ECM. This enables the encapsulation of cells,
drugs or growth factors for injection or implantation into the brain.
The primary aim of using hydrogels in stroke recovery is
to provide an exogenous ECM-based network that allows structural support
within the cerebrospinal fluid-filled cavity and promotes endogenous
brain tissue repair around the ischemic lesion. To this end, hydrogels
must have appropriate properties for the brain tissue, with the
neurovascular environment having different ECM properties compared to
other peripheral organs. Furthermore, hydrogels must be biocompatible,
without activating an immune response from the native tissue, whilst
promoting anti-inflammatory activity and recovery. Key physical
parameters for hydrogel biocompatibility include porosity, stiffness,
and preferentially the physico-chemical presence of cell adhesion
peptide (CAP) domains (72, 97, 100, 101).
Hydrogel porosity enables the diffusion of nutrients throughout the 3D
structure. If the pore size of a hydrogel is too low then nutrients and
oxygen within media may not efficiently permeate through the entire
structure, or could cause a concentration gradient; potentially leading
to necrotic regions (102, 103).
The stiffness of a hydrogel regulates the phenotype of cells, with
mechanical interactions between cells of the NVU and the ECM through
hydrostatic pressures (104) and CAP binding (105, 106), even directing the differentiation of stem cells (105–108).
Crosslinking is the mechanism by which a pre-gelation hydrogel becomes
solid, with the initiation of inter-molecular physical or chemical bonds
maintaining a 3D structure. Therefore, crosslinking dictates the
administration technique used for delivering hydrogel to the site of
injury, with injectable hydrogels requiring crosslinking (gelation) to
occur under physiological conditions [Figure 2; (109, 110)], whereas implanted hydrogels can be crosslinked in a controlled in vitro situation. Hydrogel injection has been achieved with both synthetic (43, 111, 112) and natural biopolymer hydrogels (109, 113–115) for stroke and other CNS applications.
FIGURE 2
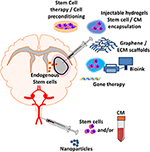
The interactions between
implanted hydrogel and endogenous brain cells have the potential to
induce many different reparative and anti-inflammatory cellular
pathways, through binding of CAPs (including RGD, IKVAV, and YISGR
motifs) to specific cell surface receptors (101).
Anti-inflammatory targets of CAPs include; cell adhesion molecules
(CAMs), which are involved in the recruitment and trafficking of
leukocytes (51, 116); integrin receptors, which in addition to having anti-inflammatory effects can have proangiogenic properties (115, 117, 118), reduce reactive gliosis (43, 119) and promote the infiltration of neural progenitor cells to the site of injury (43, 111); and growth factor receptors that can initiate similar anti-inflammatory effects (120).
CAPs can also be used to mimic growth factors and initiate preferential
cellular pathways. For example a peptide (QK) which binds to the
vascular endothelial growth factor (VEGF) receptor has been incorporated
into a hydrogel to promote angiogenesis and may also have an
anti-inflammatory effect similar to that observed after recombinant VEGF
administration (120).
Hydrogels like this VEGF-mimetic structure could aid recovery and
promote anti-inflammatory processes following stroke, and the technique
used here could be implemented for a number of growth factor mimetic
peptides to promote the desired anti-inflammatory actions.
Tissues can be decellularised to isolate the native ECM,
which has been used to create hydrogels with anti-inflammatory effects,
whilst also aiding clearance of necrotic debris and providing a
platform for regeneration through infiltration of endogenous cells to
the stroke site (100, 121).
Isolation of single ECM components for hydrogels enables the
determination of the positive or negative effects of different
biopolymers on brain tissue, with some native ECM biopolymers inducing
an anti-inflammatory response on their own; Hyaluronic acid (HA)
hydrogels in particular have been used frequently in stroke studies (43, 115, 122–126), owing to their anti-inflammatory effects through CAPs binding to CD44, which inhibits inflammation (127) as well as leukocyte rolling and extravasation through the BBB to the brain parenchyma (128).
Similarly, gelatin has been shown to exhibit native anti-inflammatory
effects in the brain following injury through repairing the BBB,
reducing circulatory molecules and cells from entering the brain
parenchyma and shifting the microglial response from neurotoxic to a
neuroreparative phenotype (129).
Implantation of hydrogels into the brain would require
invasive surgery and therefore is a higher risk regenerative strategy
than hydrogel injection and other therapeutic techniques, but does offer
certain advantages. Through use of 3D-bioprinting, a printable hydrogel
(bioink) and brain scans, an implantable structure can be created with
patient specific dimensions (Figure 2).
Anti-inflammatory and patient specific bioinks can be created with use
of a patient platelet-rich plasma (PRP)—a platelet rich fraction of
blood that contains a number of growth factors—allowing for printed
3D-anti-inflammatory structures to be implanted (130).
Certain hydrogels and bioinks require potentially toxic reagents or
ultraviolet-light to initiate crosslinking through the creation of ROS,
potentially damaging the cellular contents or initiating downstream
pro-inflammatory pathways (131).
This further highlights the need for appropriate selection of hydrogel
to ensure that anti-inflammatory effects are not negated by the
production procedure.
Hydrogels for Delivery of Anti-inflammatory Therapeutic Agents
Hydrogels can also be used as a vehicle for the delivery
of drugs, growth factors, stem cells, and NPs, to control delivery of
therapeutics over time in conjunction with the rate of hydrogel
degradation. Hydrogel degradation and gradual release of therapeutics
can be tuned to the release profile desired by modifying the physical
properties of the biopolymer, or by simply selecting a hydrogel with the
appropriate physical profile. Whilst there has been a level of success
with anti-inflammatory drugs for post-stroke recovery, the therapeutic
window and dosing strategies of these drugs could be enhanced by
encapsulation and controlled release from a hydrogel or NP structure
(Figure 2).
The half-life of drugs injected without a controlled release system is
limited, whereas when administered within a hydrogel or NP, the drug can
be present at the site of stroke damage for days or even weeks (70, 72, 125, 132).
This is a concept which can be applied to various neurodegenerative
diseases and to repairing the nervous system, justifying the
re-investigation of previously promising drugs and drug targets in a
hydrogel- or NP-based administration system (133, 134).
Controlled release has also been used in regenerative cardiology, where
the use of a hydrogel-based oxygen release system provided a sustained
release of oxygen to cardiac tissue in a model of heart failure for up
to 4 weeks, significantly reducing inflammation, ROS production and
promoting functional recovery of the damaged tissue (135).
This system could be applied to treating ischemic regions following
stroke and could allow sustained release of oxygen to promote tissue
recovery and regeneration. Hydrogels also allow for the controlled
release of NPs into the surrounding stroked tissue, for the controlled
release of anti-inflammatory NPs (45) delivering encapsulated anti-inflammatory drugs to the site of injury (44, 125).
Conditioned media are commonly produced in in vitro research, with astrocyte-derived conditioned media known to improve the survival and function of other cells of the NVU (136–138).
The potential benefit of using cell-derived conditioned media (without
cells) is to implant cell secretomes without inducing an immunogenic
response from the host tissue. This also presents the opportunity to
prime cells to secrete beneficial factors that can reduce inflammation
and promote neurorepair in the post-stroke brain (139).
Recent research has shown alterations in the secretome of mesenchymal
(stromal) stem cells (MSCs) following priming with IL-1, which promotes
the secretion of anti-inflammatory and proangiogenic growth factors that
could aid recovery (140, 141).
Similarly, encapsulation of pro-angiogenic fibroblast growth factor
(FGF)-2 within a collagen-alginate hydrogel controlled release system
has been shown to be beneficial to ischemic tissues in zebrafish models (142).
By using hydrogel controlled release systems, it is possible to
therapeutically release anti-inflammatory secretomes to aid regeneration
of damaged brain tissue.
Carbon Based Substrates
The integration of carbon-based substrates to the brain
and in hydrogels has been investigated previously for neural tissue
engineering. Two of the most commonly investigated carbon substrates for
potential stroke therapy are carbon nanotubes (CNTs) and graphene,
which have conductive properties that promote neurons and NSC activity
and survival. CNTs have been used previously for neural tissue
engineering due to their strong conductive properties, which can promote
the differentiation and function of neurons (143, 144).
CNTs, used as a substrate within hydrogels, have been used to promote
both the expression of neural phenotypes and to secrete neurotrophic
factors that could reduce inflammation (145).
The transplantation of CNTs directly into the post-stroke brain has
been shown to reduce microglial activation in the weeks following
stroke, as well as promoting neural progenitor cell differentiation to
functioning neurons (46).
The administration of CNTs before stroke also exhibited enhanced
recovery following stroke, with a reduced level of inflammatory markers (47).
Graphene is a biomaterial consisting of carbon in a 2D
plane, like the 3D structure of graphite, but with only a single-atom
thickness. For biomedical applications, graphene is commonly oxidized
(graphene oxide, GO) to make the material hydrophilic and to improve
biocompatibility (146, 147).
The structural advantage of using GO over the 3D counterpart (graphite
oxide for instance) is the enhanced surface area and hydrophilicity that
is gained from having atom-thick layers (146).
GO can be integrated into hydrogels—as a substrate or graphene
foams—for implantation after stroke, due to its mechanical, physical,
and electrical properties (148–150).
GO has been shown to have ROS scavenging and immune modulating
properties when conjugated with a synthetic hydrogel and injected into
the post-myocardial infarction heart (151),
as well as reducing neuroinflammation in a poly-ε-caprolactone scaffold
through inhibition of reactive gliosis and subsequent reduction in
glial scarring (148).
This positive immunomodulatory response shows promise for the use of GO
in hydrogel systems for stroke. More research in graphene derivatives
is needed to determine toxicity and immunogenic responses when
introduced into living systems—especially for prolonged periods of
time—before translation to humans can be considered (152).
Stem Cell Therapies
Stem cell therapy is a promising therapeutic approach in stroke and is a research priority (153).
Stem cells can differentiate into many cell types including neuronal
and endothelial lineage, and it has been widely assumed that once
implanted they may promote recovery by repopulating the necrotic cavity
present within the area of ischemic damage (154).
Indeed, several studies have tested the effect of embryonic-derived
NSC, induced pluripotent stem cells (iPSCs), embryonic stem cells
(ESCs), MSCs, and bone marrow stem cells (BMSCs) in pre-clinical stroke
models (155).
Further, the world's first fully-regulated open-label clinical trial of
neural stem cell (NSC) therapy in stroke (Pilot Investigation of Stem
Cells in Stroke, PISCES I, ReNeuron, UK), followed by the current Phase
II trial (PISCES-II) appeared safe with suggestion of functional
improvement (49), whilst autologous transplantations of MSCs in stroke patients appear safe and are associated with clinical improvement (156).
Although it has been long assumed that cell replacement is the primary
mechanism of action of implanted stem cells, a new paradigm of stem cell
actions has recently focused on their paracrine actions. It is known
that MSCs for instance exert unique therapeutic effects by secreting
anti-inflammatory and trophic factors that can transform the local
inflammatory environment when implanted locally (157), and the anti-inflammatory theory has been established for other types of stem cell (158).
To induce anti-inflammatory mechanisms, stem cells can be manipulated
or genetically edited to express certain proteins that are
neuroprotective and anti-inflammatory.
A type of anti-inflammatory cell therapy is the
transplantation of stem cells that activate downstream cellular pathways
and promote infiltration of endogenous NSC to the site of stroke injury
(Figure 2).
This involves the transplantation of stem cells which have either been
differentiated from iPSCs, ESCs, MSCs, or BMSCs to a neural progenitor
state, or are un-differentiated. The delivery of neural progenitor cells
to the site of injury triggers recovery through reducing inflammation
and reactive gliosis as well as promoting angiogenesis (159).
The transplantation of un-differentiated pluripotent stem cells (iPSCs
and ESCs) has a heightened risk of teratomas and is therefore
investigated to a lesser extent (160, 161).
In contrast, BMSCs and MSCs have been shown to have beneficial
anti-inflammatory effects through inhibition of microglia activation
without the heightened risk of tumorigenesis (162).
Further research is needed to try and optimize the transplantation of
pluripotent stem cells to avoid tumorigenic complications, with the
transplantation of cells within a hydrogel of growth factors to direct
differentiation potentially offering a better therapeutic approach.
Cell therapies are commonly administered through i.v.
injection, requiring cells to cross the BBB. The selective permeability
of the brain endothelium restricts cell infiltration resulting in much
larger doses of cell therapy being needed to have a therapeutic effect (163, 164).
To circumvent this limitation, dual therapies including stem cells
administered with biomaterial, astrocyte-derived conditioned medium or
drugs that transiently open the BBB have been considered (163, 165).
Alternatively, therapies based on administration of T-cell (Treg),
known not to cross the BBB, are able to dampen the immune response in
the brain and subsequently exert anti-inflammatory actions after stroke (166–169).
The anti-inflammatory and neuroprotective effect of Tregs occurs
through C-C Chemokine Receptor Type 5 (CCR5) interaction with the
endothelial vessel wall, which allows the Tregs to interact with
circulatory macrophages and neutrophils (167).
This information suggests CCR5 as a potential therapeutic target for
enhancing the therapeutic effect of Tregs as well as a sole target
without Treg therapy.
Studies have reported modest recovery and highlighted the
need to develop new strategies to improve the safety and efficacy of
stem cell therapies in stroke. In vitro pre-treatment of stem
cells by specific culture conditions and/or biological agents (also
known as “preconditioning” or “priming”) can improve the survival,
engraftment, immunosuppressive and paracrine properties of stem cells,
therefore enhancing their regenerative capacity. For MSCs,
preconditioning strategies have been explored in order to enhance the
anti-inflammatory properties of MSCs, including exposure to
hypoxia/growth factors (170) and inflammatory cytokines (171),
whilst the only preconditioning strategy in human stroke patients
(STARTING-2) tested the transplantation of autologous MSCs exposed to
autologous serum obtained at stroke onset (172).
Further, the encapsulation of cells within a hydrogel can create a
pre-made tissue to help promote brain repair following stroke. This
approach also improves the rate of stem cell survival from implantation,
as the cells have a support matrix to aid their integration in the host
tissue. This has been shown through using a HA based hydrogel with
growth factors, cell adhesion domains (RGD, IKVAV, and YISGR) and neural
stem cells, which enhanced stem cell survival following injection in
stroked mice (122). A similar HA has been used to inject NSC and subsequently differentiate to a neuronal lineage (123).
Gene Therapies
The mass advancements of gene-editing technologies has
enhanced the capabilities of both cell and gene therapies, with
beneficial genes being introduced to cells in vitro or in vivo
to promote the expression of neuroprotective or anti-inflammatory
factors. These advancements also raise ethical considerations as editing
of the germ line coding sequences results in permanent and hereditary
genetic changes, as opposed to editing non-germ line genes. In addition
to ethical considerations it is important to ensure that editing a
certain gene does not have off-target effects that could cause adverse
events in patients.
The use of gene therapies offers the potential to alter
cellular and molecular processes that are important to recovery from
ischemic stroke, reducing the inflammatory response and initiating
regeneration of damaged tissue. This approach has been used to deliver
anti-inflammatory gene therapies that promote production of VEGF (116, 173), anti-inflammatory neural cell adhesion molecule (NCAM) (116), or IL-1Ra (50).
These therapies were administered in rodents by intrathecal injection,
but could be improved through encapsulation within a hydrogel for
injection or implantation as this would control the release of
gene-edited cells over time to increase the therapeutic effect.
Hydrogel- and NPs-based delivery systems enable the
optimization of cell and gene therapy delivery to the site of stroke
injury. Systemically injected NPs can optimize BBB permeability through
precise surface chemistry and can be designed for controlled release of
encapsulated cells. While hydrogels must be injected directly to the
brain, they provide ECM mimetic support for both the encapsulated cells
and the surrounding host tissue. Like with NPs, the degradation profile
of the hydrogel biomaterial can enable the controlled release of cells
to the brain; both prolonging the application of anti-inflammatory
factors over time rather than having a short therapeutic effect.
Targeting genes that affect neuroinflammation has the
potential to be used as an effective therapy for multiple different
neurological diseases, with many of these diseases having an
inflammatory pathophysiology implicated in either disease onset or
progression (174, 175).
As an example, pre-clinical Alzheimer's disease research has identified
anti-inflammatory mediators that could be targeted using gene therapies
to modulate disease pathology (176–178).
In a mouse model of Alzheimer's disease, viral vectors have been used
to increase gene expression of anti-inflammatory cytokines IL-2 (178) and IL-10 (177)
which had a positive effect on pathology and cognitive function in
mice. Similar approaches to inhibit neuroinflammation have been applied
to other neurological conditions, with a multiple sclerosis gene therapy
showing neuroprotective and even disease reversing clinical outcomes in
a mouse model (179).
The principles of gene-editing that have been developed in these
neurological disease models has the potential to influence stroke gene
therapy progression, with shared inflammatory pathways in stroke
allowing for similar treatments to aid tissue regeneration in the
post-stroke brain.
Concluding Remarks
Regenerative medicine is an emerging field of
interdisciplinary research, providing potential future solutions for the
treatment of stroke and other neuroinflammatory conditions. The
efficacy of the regenerative approaches discussed in this review has
been explored mainly in pre-clinical models showing reductions in
inflammatory responses and improved recovery of brain tissue. These
pre-clinical studies form the basis of scientific evidence to progress
the translation of regenerative therapies toward clinical applications.
In particular, the use of biomaterials as anti-inflammatory agents—or as
vehicles for controlled release of anti-inflammatory agents—in the form
of NPs or hydrogels present as attractive candidates for improving the
efficacy of stroke therapies. The development and administration of
biomaterials with appropriate physical properties to treat post-stroke
inflammation is crucial; with additional complexities and potential
advantages being acquired from the bioprinting of implantable tissues.
Overall the vast array of NPs, hydrogels, and cell and gene therapies
being investigated for the treatment of stroke is very promising and may
lead to the licensing of a regenerative medicine inspired treatment in
the years to come.
No comments:
Post a Comment